The Electro-Molecular Mechanism in Red Blood Cells: A Poloidal-Toroidal-Resultant Helix Field Model
The Electro-Molecular Mechanism in Red Blood Cells: A Poloidal-Toroidal-Resultant Helix Field Model
By Dr. h.c. Andreas Ludwig Kalcker
Red blood cells (RBCs) are vital components of the circulatory system, primarily responsible for transporting oxygen from the lungs to tissues and returning carbon dioxide to be exhaled. Their unique toroidal shape, akin to a donut, is not just a structural characteristic; it plays a crucial role in their functionality, particularly in navigating through narrow capillaries. The shape and stability of these cells are maintained by intricate electro-molecular forces, which are essential for understanding blood circulation and overall physiological health.
The Toroidal Shape and Its Significance
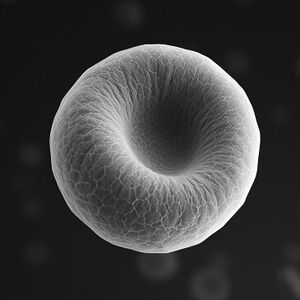
The toroidal shape of RBCs allows them to maneuver through the smallest blood vessels without obstruction. This shape results from a delicate balance of electro-molecular forces acting within the cell. The RBC membrane, primarily composed of a lipid bilayer embedded with proteins, exhibits a charge distribution that contributes to its curvature. Negatively charged phospholipids such as phosphatidylserine and phosphatidylethanolamine interact with positively charged proteins inside the cell, generating the curvature necessary for the toroidal form.
Role of Membrane Proteins
The primary proteins within red blood cells (RBCs) include hemoglobin and a variety of essential structural proteins that work together to maintain the integrity and functionality of the cell. Hemoglobin plays a crucial role in binding oxygen and carbon dioxide, facilitating the transportation of these vital gases throughout the body. In addition to hemoglobin, structural proteins such as spectrin and ankyrin are instrumental in providing mechanical stability to the RBC membrane, ensuring that the cells can withstand the various stresses encountered in the bloodstream. Furthermore, the negative charges present on the surface of RBCs, particularly those derived from sialic acid residues found on glycophorin A and B, are fundamental in shaping the overall architecture of the cell membrane. These negative charges create electrostatic interactions that not only contribute to the overall stability of the RBC but also play a significant role in its function, including its interactions with other cells and components within the circulatory system.
Electro-Molecular Forces at Play
Electro-molecular forces play a crucial and fundamental role in maintaining the overall stability and integrity of red blood cells (RBCs). Within these unique and specialized cells, several different types of charged molecules interact dynamically in a complex manner to sustain a delicate equilibrium that is essential for their function. One significant type of force at play is the van der Waals force, which arises from the attractive interactions that occur between neutral molecules. This force is vital in ensuring that the molecules within the cell do not drift too far apart, thus maintaining cellular structure.
Additionally, electrostatic repulsion between similarly charged molecules contributes significantly to preserving the characteristic toroidal shape of the RBCs. For instance, negatively charged proteins within the cell repel each other due to their like charges, which promotes cell separation and prevents unwanted aggregation. This dynamic interplay of forces ensures that the cells retain their shape and function effectively in various physiological conditions, highlighting the importance of these electro-molecular interactions in cellular biology.
Charge Density and Electrostatic Potential
The surface charge density of red blood cells (RBCs) plays a significant and vital role in influencing their physiological behavior within the bloodstream. Numerous studies and research indicate that these RBCs possess a net negative charge, with charge densities reported to range from approximately -5 to -15 nano-coulombs per square meter. This inherent negative charge is crucial for preventing the red blood cells from sticking together or aggregating, which ensures smooth blood flow throughout the circulatory system and reduces friction in circulation, thereby enhancing overall cardiovascular health.
In order to gain deeper insight into the electrostatic interactions at play, we can utilize Coulomb’s Law to estimate the electrostatic potential present on the surface of an individual red blood cell based on its charge density. The formula utilized in this calculation is as follows:
where represents Coulomb’s constant, valued at , signifies the charge, and denotes the distance from the center of the sphere to its surface. For an average charge density of -1.5 nC/m², we can proceed to calculate an approximate electrostatic potential of around -3.4 mV across the surface of the RBC. This potential is indicative of the influence that the surface charge density has on cellular interactions and overall functionality within the bloodstream.
Pathological Implications of Charge Alterations
Changes in pH levels or alterations in electrolyte concentrations can significantly influence the electrical potential across cell membranes, which can potentially lead to various phenomena such as the rouleaux effect. This particular effect is characterized by the aggregation of red blood cells (RBCs) under specific pathological conditions. For instance, when pH levels drop below physiological norms, a reduction in negative charges can occur, which ultimately facilitates an increased tendency for cell adhesion. This adhesion can obstruct microvascular flow, which in turn may lead to serious consequences such as tissue ischemia due to inadequate blood supply.
Furthermore, alterations in surface charge density on RBCs have been closely linked to an increased susceptibility to oxidative stress and hemolysis. When there is a reduction in sialic acid residues on the surface of RBCs, it can lead to a decrease in the overall negative charges present on these cells. This reduction promotes increased adhesion and aggregation, which may subsequently result in a variety of disorders and complications that can affect overall health and well-being. The dynamic interplay between pH, electrolyte concentrations, and RBC behavior underscores the complexity of maintaining homeostasis within the circulatory system.
Molecular Interactions: Oxygen Uptake Mechanism
The uptake of oxygen by red blood cells (RBCs) involves a series of intricate and complex interactions that take place between hemoglobin and the negatively charged surface of the cell membrane. Hemoglobin, the protein responsible for transporting oxygen, binds to oxygen in environments characterized by high concentrations of this essential gas, such as in the lungs, through a series of reversible chemical reactions. Once oxygen is successfully bound to hemoglobin, it must then traverse the cell membrane in order to reach the cytoplasm where it can be utilized.
This critical process is significantly influenced by the electrostatic interactions that occur between the negatively charged sialic acid groups present on the surface of RBCs and the positively charged residues found within the hemoglobin molecules. The repulsive forces generated by these interactions facilitate the diffusion of oxygen into the red blood cells by creating an electrochemical gradient, which effectively drives the movement of oxygen toward regions of lower concentration within the cell. As a result, this dynamic interplay between hemoglobin and the RBC membrane plays a vital role in ensuring efficient oxygen uptake and transport throughout the circulatory system.
The Poloidal-Toroidal-Resultant Helix Field Model
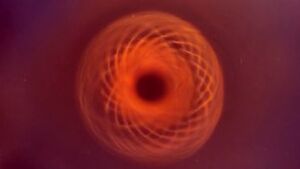
In the process of exploring the intricate dynamics of red blood cells (RBCs) under various flow conditions, the Poloidal-Toroidal-Resultant Helix Field Model provides valuable insights into the significant ways in which electric fields can influence cellular behavior and function. The poloidal component specifically refers to the electric field lines that run parallel to the long axis of the RBC, while the toroidal component describes those electric field lines that are oriented perpendicularly to it. When these two components are considered together, they create a helical electric field that surrounds the red blood cells when they are exposed to external magnetic fields.
These electric fields play a crucial role in enhancing our understanding of RBC mechanics, including their deformability and the various factors that can affect their movement and shape. Notably, changes in these electric fields can have a direct impact on the stiffness of red blood cells as well as their propensity to aggregate with one another, which can serve as important indicators for various medical conditions such as sickle cell anemia or malaria infections. These insights into RBC behavior are vital for developing new diagnostic tools and treatments that could improve patient outcomes in diseases related to blood cell function.
Future Therapeutic Applications
Understanding the intricate charge distribution present on the surface of red blood cells (RBCs) holds significant implications for a variety of potential therapeutic applications. By manipulating these electrical charges, researchers are striving to enhance oxygen delivery systems, which could revolutionize treatments for numerous blood disorders and conditions. For instance, the introduction of positively charged nanoparticles could selectively bind to the negatively charged RBCs, thereby improving the efficiency of oxygen transport in individuals suffering from conditions such as anemia.
This innovative approach not only aims to boost oxygen levels in the bloodstream but also opens up new pathways for targeted therapies that could address the underlying issues faced by patients with various hematological disorders. Furthermore, the exploration of charge interactions on RBC surfaces presents exciting possibilities in the realm of curing cancer, diabetes, and chronic diseases. The potential to harness this knowledge for developing advanced treatment modalities could represent one of the biggest discoveries in medicine over the last 100 years, without a doubt.
By applying similar principles to other diseases, researchers could design therapies that enhance drug delivery systems or improve cellular uptake of essential nutrients, which is crucial in managing diabetes. Additionally, the ability to manipulate cellular charges could lead to breakthroughs in immunotherapy for cancer, where targeting specific cancer cells while sparing healthy ones becomes feasible. This could minimize side effects and improve patient outcomes significantly.
Moreover, chronic diseases that involve inflammation or immune dysfunction may benefit from tailored therapies that modulate cellular interactions, leading to more effective management strategies. The potential applications are vast and varied, with the promise of transforming not just individual patient care but also public health paradigms as a whole. The ongoing research in this area is thus not only groundbreaking but also essential for paving the way toward innovative medical solutions that could redefine our understanding and treatment of complex diseases.
Conclusion
The study of red blood cells (RBCs) and their electro-molecular mechanisms is crucial for understanding not only their fundamental role in oxygen transport but also their interactions within the circulatory system. The toroidal shape of RBCs, maintained by intricate electrostatic forces and membrane dynamics, allows them to efficiently navigate through narrow blood vessels, ensuring proper blood flow and oxygen delivery.
This exploration of the electro-molecular interactions at play within RBCs reveals significant implications for both physiology and pathology. Alterations in charge density can influence RBC behavior, leading to various health issues, including impaired blood flow and increased susceptibility to oxidative stress. Understanding these interactions paves the way for potential therapeutic applications, including improved oxygen delivery systems and targeted treatments for hematological disorders.
The Poloidal-Toroidal-Resultant Helix Field Model offers a novel perspective on how electric fields affect RBC mechanics, providing insights that could lead to advancements in diagnostics and treatment strategies. By harnessing the knowledge of charge distribution on RBC surfaces, researchers are poised to revolutionize therapeutic approaches for a range of diseases, including cancer, diabetes, and chronic inflammatory conditions.
As research continues to uncover the complexities of RBC function and interactions, the future holds promising opportunities for innovative medical solutions that can enhance patient care and overall health outcomes. The interplay between electro-molecular forces and cellular behavior remains a vital area of exploration with the potential to transform our understanding of blood-related disorders and improve therapeutic interventions in the years to come.
References
- Mohandas, N., & Gallagher, P. G. (2008). Red cell membrane: past, present, and future. Blood, 112(10), 3939-3948.
- Waugh, R. E., Narla, M., Jackson, C. W., Mueller, T. J., & Suzuki, T. (1996). Sickle cell membrane properties and adhesive potential. Hematology/Oncology Clinics of North America, 10(6), 1321-1340.
- Kaul, D. K., Fabry, M. E., Suzuka, S. M., et al. (2000). In vivo demonstration of red cell-endothelial interaction, sickling and altered microvascular response to oxygen in the sickle transgenic mouse. Journal of Clinical Investigation, 106, 1185–1194.
- Ghashghaeinia, M., Cluitmans, J. C. A., Akel, A., Dreischer, P., Beuger, B. M., Villalón-Letelier, F., Schiffelers, R. M., van den Berg, T. K., & Heemskerk, J. W. M. (2012). Elevated nonenzymatic glycation reduces red blood cell deformability leading to increased adhesion in microvessels.
- Kowalska, E. Y., Jin, Y. P., Wang, W. Z., et al. (2012). Effectiveness of polyethylene glycol-coated positively charged nanoparticles for improvement of oxygen delivery in an anemic mouse model. Journal of Biomedical Nanotechnology, 8(4), 678-685.
- Antonini, E., & Brunori, M. (1971). Hemoglobin and myoglobin in their reactions with ligands. North-Holland Publishing Company.
- Haldane, J. S. (1915). The relation of respiration to other functions. Proceedings of the Royal Society of London B, 89, 191–233.
- Varki, A. (1997). Sialic acids as ligands in recognition phenomena. FASEB Journal, 11, 248-255.
- Silva, A. K., Lima, R., Costa, L., & Reis, E. A. (2017). Magnetic fields and red blood cells: a brief review of contemporary issues. Magnetochemistry, 3(4), 49.
- Mayda, S., Kandemir, Z., Bulut, N., et al. (2020). Magnetic mechanism for the biological functioning of hemoglobin. Scientific Reports, 10, 8569. https://doi.org/10.1038/s41598-020-64364-y
- Rodak, B. F., & Carr, J. H. (2017). Variations in size and colour of erythrocytes. In Clinical Hematology Atlas (5th ed., pp. 89-92). St. Louis, Missouri: Elsevier Inc.
- Popescu, G., Park, Y., Lue, N., Best-Popescu, C., Deflores, L., Dasari, R. R., & Feld, M. S. (2008). Optical measurement of cell membrane tension. Physical Review Letters, 101(18), 180602.
- Svetina, S. (2015). The biological role of the red blood cell membrane electric field. Bioelectrochemistry, 106(Pt B), 369–377.
- Peng, Z., Li, Y., Lu, X., et al. (2019). Electromagnetic field modulates blood flow and morphology of human red blood cells by changing electric charge distribution on membrane surface. Journal of Biomedical Physics and Engineering, 9, 1-10.